Imagine your cell as a miniature city bustling with activity. Thousands of chemical reactions are constantly happening, transforming raw materials into energy, building blocks, and everything else life requires. How is this chaotic symphony orchestrated with precision? Metabolic pathways are the answer. Think of them as highly organized assembly lines, each step managed by a specialized worker: an enzyme.
These pathways aren’t just random reaction sequences. They’re crucial for every biological process, from generating the energy you need to think right now to building complex molecules. Understanding their structure and control is fundamental to grasping how life works at the molecular level.
What Are Metabolic Pathways?
Simply put, a metabolic pathway is a series of interconnected, enzyme-catalysed reactions. The product of one reaction often becomes the reactant (substrate) for the next, creating a chain or cycle of transformations. This multi-step approach allows for much finer control and regulation compared to a single, large reaction.
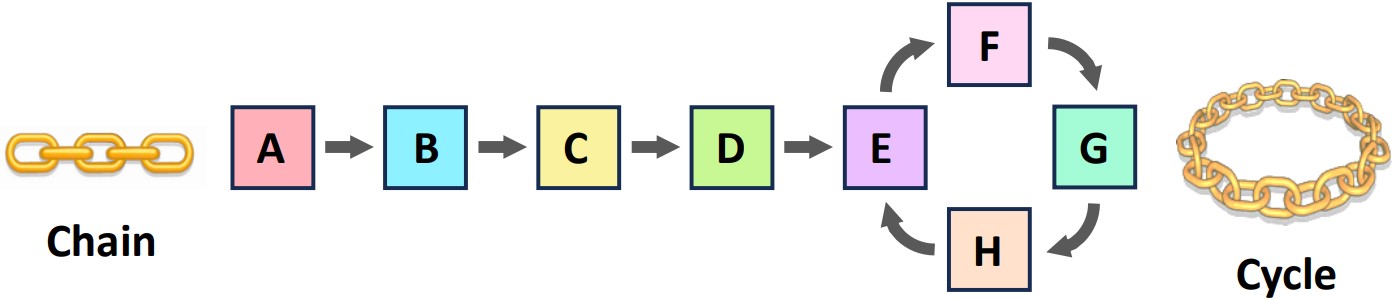
Location, Location, Location: Intracellular vs. Extracellular Pathways (IB C1.1.11)
Metabolic action happens both inside and outside the cell:
Intracellular Pathways: These occur within the cell, often confined to specific organelles.
Example: The Calvin cycle, where carbon dioxide is converted into glucose using light energy, takes place inside the chloroplasts of plant cells. Another example is the Krebs cycle within mitochondria during cellular respiration.
Extracellular Pathways: These reactions happen outside the cell boundary.
Example: The breakdown of large food molecules like proteins, carbohydrates, and fats in your digestive system. Enzymes secreted from cells (like salivary amylase in your mouth or pepsin in your stomach) work outside the cells to hydrolyse these complex substances into smaller, absorbable units.
Structure Matters: Linear vs. Cyclical Pathways (IB C1.1.13)
Metabolic pathways can be organized in two main structural formats:
Linear Pathways: These pathways proceed in a straight line, with an initial substrate being converted through a series of steps into a final product.
Example: Glycolysis, the initial stage of cellular respiration, is a linear pathway converting glucose into pyruvate. Another example is the synthesis of a specific amino acid through a series of modifications of a precursor molecule.
Cyclical Pathways: In these pathways, a molecule enters the cycle, is modified through a series of reactions, and is regenerated at the end to begin the cycle again. Intermediate products are constantly being transformed within the loop.
Example: The Krebs cycle (also known as the Citric Acid Cycle) is a classic example. Acetyl-CoA enters, undergoes oxidation, releasing CO2 and energy carriers, and the starting molecule (oxaloacetate) is regenerated. The Urea cycle in the liver is another cyclical pathway.
Why the Multi-Step Approach? Regulation is Key
Metabolic pathways provide a sophisticated level of regulation. Because each step involves a specific enzyme and intermediate product, the cell can control the rate of the overall pathway by influencing individual enzymes or the availability of intermediates at various points. This allows for precise control over cellular processes, ensuring resources aren’t wasted and products are made only when needed.
Energy Transformations: The Inevitable Heat (IB C1.1.12)
Life requires constant energy transformations. Whether breaking down glucose (catabolism) or building proteins (anabolism), these metabolic reactions involve converting energy from one form to another. However, these conversions are never 100% efficient. According to the principles of thermodynamics (specifically the Second Law, which you might encounter in physics or chemistry), some energy is always lost as unusable heat.
This heat production from metabolic activity is actually crucial for some organisms. Endotherms (like mammals and birds) actively use this metabolic heat to maintain a stable, constant internal body temperature, regardless of the external environment. They can regulate their metabolic rate – increasing it through activity or shivering to generate more heat, or decreasing it (e.g., entering a state of torpor or hibernation) to conserve energy and reduce heat loss when resources are scarce or temperatures are extreme. This metabolic heat generation is simply a byproduct of the essential biochemical reactions keeping them alive.
Pathway Traffic Control: Enzyme Inhibitors
Just as traffic lights and road closures manage vehicle flow, cells need ways to control the rate and direction of metabolic pathways. Enzyme inhibitors are molecules that play this crucial regulatory role. An inhibitor is a molecule that binds to an enzyme and reduces or prevents its activity.
Inhibitors can originate within the cell itself, acting as natural regulators to fine-tune metabolic rates. They can also come from outside, like toxins, venoms, or drugs designed to target specific enzymes in pathogens or disease processes.
Enzyme inhibition is a fundamental concept in:
Metabolic Regulation: Cells use inhibitors to switch pathways on or off as needed.
Pharmacology: Many drugs function as enzyme inhibitors to treat diseases (e.g., antibiotics, cancer therapies, cholesterol-lowering medications).
Toxicology: Poisons often work by inhibiting essential enzymes.
Inhibitors are often classified based on how they interact with the enzyme and whether their effect is permanent.
Reversible vs. Irreversible Inhibition
Reversible Inhibition: The inhibitor binds to the enzyme via weak, non-covalent bonds (like hydrogen bonds or ionic interactions). The inhibitor can detach from the enzyme, allowing enzyme activity to resume. This type of inhibition is in dynamic equilibrium.
Irreversible Inhibition: The inhibitor forms strong, permanent covalent bonds with the enzyme. This binding permanently alters or blocks the enzyme’s active site, rendering it inactive. The only way to regain activity is to synthesize new enzyme molecules.
Major Types of Enzyme Inhibition
Now let’s look at specific mechanisms (and link them back to the IB Biology syllabus!):
Competitive Inhibition
Mechanism: A competitive inhibitor is a molecule that is structurally similar to the enzyme’s natural substrate. It competes with the substrate for the active site of the enzyme. Both the substrate and the inhibitor cannot bind to the active site simultaneously.
Competitive Inhibition Diagram Source: Bio Ninja Effect: When the inhibitor is bound, it physically blocks the substrate from accessing the active site, thus preventing catalysis.
Reversibility: Competitive inhibitors typically bind reversibly via weak bonds.
Overcoming Inhibition: Increasing the concentration of the substrate can overcome competitive inhibition. By flooding the system with substrate, you statistically increase the chance that a substrate molecule will bind to the active site before an inhibitor molecule does. Imagine a popular ride at an amusement park: the inhibitor is someone trying to cut the line (bind to the active site), but if there are thousands of people in the line (substrate), the line-cutter has a much lower chance of getting on the ride first.
Example: Consider an enzyme that converts molecule X into molecule Y. A competitive inhibitor Z might look very similar to X and bind to the enzyme’s active site. If you increase the amount of X, X is more likely to bind and the reaction proceeds, reducing the impact of Z. A new example: A certain type of herbicide works by mimicking a plant enzyme’s substrate involved in amino acid synthesis. It binds to the enzyme’s active site, blocking the plant’s ability to make essential amino acids, thus killing the plant.
Non-Competitive Inhibition
Non-Competitive Inhibition Diagram Source: Bio Ninja Mechanism: A non-competitive inhibitor binds to a site on the enzyme that is different from the active site. This alternative site is called the allosteric site. Binding at the allosteric site causes a conformational change (a change in shape) in the enzyme molecule, which in turn alters the shape of the active site.
Effect: The active site’s altered shape means the substrate can no longer bind effectively, or if it does bind, the enzyme can no longer catalyze the reaction efficiently. The enzyme is essentially disabled, regardless of whether the active site is occupied.
Reversibility: Non-competitive inhibition can be either reversible or irreversible, depending on the type of bond formed at the allosteric site.
Overcoming Inhibition: Increasing the substrate concentration does not overcome non-competitive inhibition. Since the inhibitor isn’t competing for the active site, flooding the system with substrate won’t restore the active site’s correct shape. Using the amusement park analogy: the inhibitor isn’t cutting the line; they’ve sabotaged the ride’s mechanism so it can’t function, no matter how many people are waiting!
Example: Imagine a crucial enzyme in nerve cells. A toxin might bind to an allosteric site on this enzyme, changing the active site’s shape and preventing it from processing a key neurotransmitter. Increasing the neurotransmitter level wouldn’t help because the enzyme is structurally impaired. A new example: Heavy metal ions (like lead or mercury) can often act as non-competitive inhibitors by binding to sulfhydryl (-SH) groups in cysteine residues found away from the active site in many enzymes. This disrupts the protein’s overall 3D structure, rendering the active site non-functional.
Feedback Inhibition
Feedback Inhibition Diagram Source: Bio Ninja Mechanism: Feedback inhibition is a vital regulatory mechanism, usually involving non-competitive inhibition. In this system, the final product of a metabolic pathway acts as an inhibitor for an enzyme earlier in the same pathway (often the first committed step). The product typically binds to an allosteric site on the early-pathway enzyme.
Effect: As the concentration of the final product increases, more product molecules bind to the initial enzyme, inhibiting its activity. This slows down or stops the entire pathway, preventing the overproduction of the product and conserving resources (like the initial substrates and intermediates).
It’s a Negative Feedback Loop: The increase in the product inhibits its own production – a classic example of negative feedback maintaining homeostasis.
Example: The synthesis of the amino acid isoleucine from threonine in bacteria is a classic example (IB Example). This involves a multi-step pathway. When sufficient isoleucine has been synthesized, isoleucine molecules bind to an allosteric site on the first enzyme in the pathway (threonine deaminase). This inhibits the enzyme, stopping the conversion of threonine and thus halting the production of more isoleucine. This mechanism prevents the cell from wasting valuable threonine when isoleucine levels are high.
Mechanism-Based Inhibition
Mechanism: This is a special type of irreversible inhibition. The inhibitor molecule often structurally resembles the substrate and binds to the enzyme’s active site. The enzyme begins its normal catalytic process on the inhibitor molecule. However, during this process, the inhibitor is chemically modified by the enzyme, and this modified inhibitor then forms a strong, permanent covalent bond with the enzyme’s active site.
Effect: The enzyme is permanently inactivated because the inhibitor is now covalently attached to its active site, preventing any substrate from binding or catalysis from occurring. The enzyme essentially participates in its own inactivation, hence the term “suicide inhibition.”
Reversibility: This inhibition is irreversible.
Example: Penicillin is a famous example. It inhibits bacterial enzymes called transpeptidases (also known as Penicillin-Binding Proteins or PBPs) which are essential for building the rigid peptidoglycan layer of the bacterial cell wall. Penicillin structurally mimics the end part of the peptide chain that the enzyme normally links together. The transpeptidase binds penicillin to its active site and attempts to catalyze a reaction on it. In the process, penicillin forms a covalent bond with a serine residue in the active site, permanently deactivating the enzyme. Without functional cell wall synthesis, the bacterial cell wall weakens, and internal osmotic pressure causes the bacterium to swell and lyse (burst). This is why penicillin is a highly effective antibiotic – it targets a structure (peptidoglycan) and enzymes essential only in bacteria, minimizing harm to human cells. However, bacteria can develop resistance, often by mutating their transpeptidases so penicillin can’t bind effectively, or by producing enzymes (like beta-lactamase) that break down penicillin before it can reach the target enzyme.
Connecting the Dots
Understanding enzymes, metabolic pathways, and their regulation via inhibition is fundamental to many areas of biology. It connects to:
Cellular Respiration & Photosynthesis: These complex pathways are prime examples of linear and cyclical processes involving numerous enzymes and points of regulation.
Genetics: Gene expression controls the synthesis of enzymes, directly impacting metabolic capabilities. Mutations can alter enzyme structure and function, leading to metabolic disorders or drug resistance.
Physiology: Hormones and signals often regulate metabolic pathways to maintain homeostasis (e.g., insulin regulating glucose metabolism).
Biotechnology & Medicine: Many drugs target enzymes to treat diseases, and understanding enzyme kinetics and inhibition is critical for drug design.
FAQ: Your Burning Questions Answered!
Competitive inhibitors bind at the active site (competing with the substrate), while non-competitive inhibitors bind at an allosteric site (causing a conformational change to the active site). Competitive inhibition can be overcome by increasing substrate concentration, but non-competitive inhibition cannot.
While feedback inhibition often involves the product binding to an allosteric site (making it non-competitive), it’s the regulatory mechanism of the product inhibiting an early enzyme that defines it as feedback inhibition. Most textbook examples, like isoleucine synthesis, show the product binding to an allosteric site.
Reversible inhibition is temporary. If the concentration of the inhibitor decreases (e.g., it’s metabolized or transported away), or if the concentration of the substrate increases (in the case of competitive inhibition), the inhibitor will detach from the enzyme, and the enzyme will regain its full activity.
Irreversible inhibitors permanently disable the enzyme target. This means a single inhibitor molecule can knock out one enzyme molecule forever. This makes them potent, especially when targeting essential enzymes in pathogens (like the bacterial cell wall synthesis enzymes). However, irreversibility can also make them more toxic if they accidentally target essential enzymes in the host organism.
It’s incredibly useful! Many drugs are designed as enzyme inhibitors. For example, statins inhibit an enzyme in cholesterol synthesis, certain cancer drugs inhibit enzymes needed for cell division, and many antibiotics inhibit enzymes essential for bacterial survival (like penicillin targeting cell wall synthesis). By selectively inhibiting specific enzymes, we can disrupt disease processes.
Absolutely! Temperature and pH affect enzyme structure and activity. They can influence how well an inhibitor binds and how effectively the enzyme functions overall. Extreme temperatures or non-optimal pH can denature the enzyme, inactivating it permanently (which is different from specific inhibition mechanisms, though the end result is loss of activity). Within optimal ranges, changes in T or pH can alter binding affinities of both substrate and inhibitor.